 |
Information box |
The main purpose of this site is to extend the
intraoperative monitoring to include the neurophysiologic
parameters with intraoperative navigation guided with Skyra 3
tesla MRI and other radiologic facilities to merge the
morphologic and histochemical data in concordance with the
functional data.
CNS Clinic
Located in Jordan Amman near Al-Shmaisani hospital, where all
ambulatory activity is going on.
Contact: Tel: +96265677695, +96265677694.
Skyra running
A magnetom Skyra 3 tesla MRI with all clinical applications
started to run in our hospital in 28-October-2013.
Shmaisani hospital
The hospital where the project is located and running diagnostic
and surgical activity. |
|
 |
|
|
 |
Introduction |
 |
Magnetic resonance spectroscopy (MRS) is used to measure the
levels of different metabolites in body tissues. The MR signal
produces a spectrum of resonances that correspond to different
molecular arrangements of the isotope being "excited". This
signature is used to diagnose certain metabolic disorders,
especially those affecting the brain, and to provide information
on tumor metabolism.
Magnetic resonance spectroscopic imaging (MRSI) combines both
spectroscopic and imaging methods to produce spatially localized
spectra from within the sample or patient. The spatial
resolution is much lower (limited by the available SNR), but the
spectra in each voxel contains information about many
metabolites. Because the available signal is used to encode
spatial and spectral information, MRSI requires high SNR
achievable only at higher field strengths (3 T and above).
 |
What we see in
spectroscopy |
 |
Because of the relatively low sensitivity of
in vivo MRS, in order for a compound to be detectable, generally
its concentration must be in the millimolar range, and it must
be a small, mobile molecule. Large and/or membrane-associated
molecules are not usually detected, although they may exhibit
broad resonances that contribute to the baseline of the
spectrum. The information content of a proton brain spectrum
depends on quite a few factors, such as the field strength used,
echo time, and type of pulse sequence. At the commonly used 1.5
T field strength, at long echo times (e.g. 140 or 280 ms are
often used;) only signals from Cho, Cr, and NAA are observed in
normal brain, while compounds such as lactate, alanine, or
others may be detectable if their concentrations are elevated
above normal levels due to pathological processes.
At short echo times (e.g. 35ms or less) compounds with shorter
T2 relaxation times (or multiplet resonances which become
dephased at longer echo times) also become detectable. These
include resonances from glutamate, glutamine, and GABA, which
are not resolved from each other at 1.5 T, myo-inositol, as well
as lipids and macromolecular resonances.
Spectral appearance at 3.0T is generally similar to that at
1.5T, although the coupling patterns of the multiplet resonances
are somewhat different. Most of the multiplets (e.g. Glu, Gln,
mI, taurine) are strongly coupled at these field strengths, and
Glu and Gln overlap slightly less at 3 T than at 1.5 T. As field
strengths increase further, to 4.0 and 7.0 T, spectral
resolution progressively increases (provided that magnetic field
homogeneity can be maintained) and more compounds can be
assigned with confidence, including separating N-acetyl aspartyl
glutamate (NAAG) from NAA, separation of Glu from Gln, and the
detection of up to 14 different compounds at short echo times at
7T. A complete list of metabolite structures, chemical shift,
coupling constants, and a summary of all compounds that have
been detected in the human brain by proton MRS is
given in Table 1. For more details about metabolite map in the
brain, click here!
NAA
The largest signal in the normal adult brain spectrum, the
acetyl group of N-acetyl aspartate resonates at 2.01 ppm, with a
usually unresolved (except at very high fields) contribution
from N-acetyl aspartyl glutamate (NAAG) at 2.04 ppm. The
aspartyl group also exhibits a pH-sensitive, strongly coupled
resonance at approximately 2.6 ppm. Despite being one of the
most abundant amino acids in the central nervous system, NAA was
not discovered until 1956, and its function has been the subject
of considerable debate. It has been speculated to be a source of
acetyl groups for lipid synthesis, a regulator of protein
synthesis, a storage form of acetyl-CoA or aspartate, a
breakdown product of NAAG (which, unlike NAA, is a
neurotransmitter), or an osmolyte. NAA is believed to be
synthesized in neuronal mitochondria, from aspartate and
acetyl-CoA. NAA is often referred to as a neuronal marker, based
on several lines of evidence. For instance, immunocytochemical
staining techniques have indicated that NAA is predominantly
restricted to neurons, axons, and dendrites within the central
nervous system; and studies of diseases known to involve
neuronal and/or axonal loss (for instance, infarcts, brain
tumors, or multiple sclerosis (MS) plaques) have without
exception found NAA to be decreased. In pathologies such as MS,
good correlations between brain NAA levels and clinical measures
of disability have been found, suggesting that higher NAA levels
may be associated with better neuronal function. Animal models
of chronic neuronal injury have also been shown to give good
correlations between NAA levels (as measured by MRS) and in
vitro measures of neuronal survival. All of these studies
therefore suggest that MRS measurements of NAA may be useful for
assessment of neuronal health or integrity in the central
nervous system.
However, other experiments suggest that caution should be used
in interpreting NAA solely as a neuronal marker. For instance,
it has also been reported that NAA may be found in non-neuronal
cells, such as mast cells or isolated oligodendrocyte
preparations, suggesting that NAA may not be specific for
neuronal processes. It is unclear if these cells are present at
high enough concentrations in the normal human brain to
contribute significantly to the NAA signal, however. There are
also some rare cases where NAA metabolism is perturbed, almost
certainly independently of neuronal density or function. One
example is the leukodystrophy, Canavan’s disease, which is
associated with a large elevation of intracellular NAA, owing to
deficiency of aspartoacylase, the enzyme that degrades NAA to
acetate and aspartate.
In addition, there has been a case report of a child, with
mental retardation, with a complete absence of NAA. This case
suggests that neurons can exist without the presence of NAA, and
indeed that NAA is not necessary for neuronal function. While
these observations indicate that there is evidence both for and
against NAA as a measure of neuronal density and function, on
balance, NAA does appear to be one of the better surrogate
neuronal markers that can be measured non-invasively in humans.
Like all surrogate markers, there will be occasions when it does
not reflect the true neuronal status.
Decreases in NAA in some diseases have been shown to be
reversible, suggesting that low NAA does not always indicate
permanent neuronal damage. Reversible NAA deficits (either
spontaneous, or in response to treatment) have been observed in
diseases such as multiple sclerosis, mitochondrial diseases,
AIDS, temporal lobe epilepsy, amyotrophic lateral sclerosis, or
acute disseminated encephalomyelitis (ADEM). Therefore, in
individual patients, while a low NAA signal in some pathologies
may indicate irreversible neuroaxonal damage (e.g. strokes,
brain tumors), in others it may be due to dysfunction (perturbed
NAA synthesis or degradation) that may be reversible with either
treatment-related or spontaneous recovery.
Choline
The choline signal (Cho, 3.20 ppm) is a composite peak
consisting of contributions from the trimethyl amine (–N(CH3)3)
groups of glycerophosphocholine (GPC), phosphocholine (PC), and
a small amount of free choline itself. These compounds are
involved in membrane synthesis and degradation, and it has often
been suggested that they are elevated in disease states where
increased membrane turnover is involved (e.g. tumors). Glial
cells have also been reported to have high levels of Cho. Other
pathological processes which lead to Cho elevation include
active demyelination, either resulting from the degradation of
myelin phospholipids primarily to GPC, or perhaps due to
inflammation. Elevated Cho levels seem to be a characteristic of
many types of neoplasms, including high-grade brain tumors
(provided that they are not necrotic), prostate, breast, head
and neck, and other tumors. In particular, it would appear that
malignant transformation of tumors involves an increase in PC
relative to GPC.
Low brain Cho has been observed in hepatic encephalopathy, and
there is also some evidence to suggest that dietary intake of
choline can modulate cerebral Cho levels. In both cases, this
may be due to altered (decreased or increased) systemic
transport of Cho to the brain. Cho also shows quite strong
regional variations in the brain, usually with somewhat higher
levels in white matter than gray, although the thalamus,
hypothalamus, and insular cortex also show high levels in the
normal brain.
Creatine
The creatine methyl resonance (Cr, 3.03 ppm) is a composite peak
consisting of both creatine and phosphocreatine, compounds that
are involved in energy metabolism via the creatine kinase
reaction, generating ATP. In many spectra, a second resonance
from the CH2 of creatine is also observed at 3.91 ppm (provided
that it is not saturated by the water-suppression pulses). In
vitro, glial cells contain a two- to fourfold higher
concentration of creatine than do neurons. Creatine also shows
quite large regional variations, with lower levels in white
matter than gray matter in normal brain, as well as very high
levels of Cr in the cerebellum compared to supratentorial
regions.
Since creatine is synthesized in the liver and transported to
the brain, chronic liver disease leads to lower cerebral
creatine concentration. There is also a rare group of diseases
which involve total Cr deficiency in the brain, resulting from
either a lack of synthesis in the liver (GAMT, guanidinoacetate
methyl transferase deficiency) or defective transport to the
brain.
Lactate
In the human brain, the lactate methyl resonance (1.31 ppm) is
below (or at the very limit of) detectability in most studies,
due to the low concentration of lactate within the brain under
normal conditions. A small lactate signal may sometimes be
observed in ventricular cerebrospinal fluid (CSF), where it is
more visible due to either being present in higher concentration
(than brain), or because it has a longer T2 relaxation time.
Lactate is often increased and detected by MRS in pathological
conditions; lack of oxygen (due to either hypoxia or ischemia)
will cause an increase in lactate when metabolism of glucose
through the Krebs cycle can no longer be sustained. Therefore,
increased levels of brain lactate have been observed using MRS
in a variety of conditions, including both acute and chronic
ischemia, and hypoxia (where it is a poor prognostic indicator).
Also, defects in the Krebs cycle (even in the presence of
oxygen) can cause lactate to become elevated. Some examples of
pathologies where this may occur include brain tumors,
mitochondrial diseases, and other conditions. Small elevations
of lactate have also been reported in the visual cortex during
photic stimulation, believed to be due to increased
non-oxidative glycolysis, but this effect does not appear to be
particularly reproducible. Lactate may also be difficult to
distinguish from overlapping lipid resonances, either
originating from the brain itself, or spatial contamination from
the very strong lipid signals in the scalp. Several approaches
can be used to distinguish lactate from lipid, including the use
of spectral editing techniques, although one of the simplest
ways is to use an echo time of approximately 140 ms (1/J, where
J ≈ 7 Hz) where the lactate methyl resonance should be inverted.
Myo-inositol
One of the larger signals in short echo time spectra occurs from
myo-inositol (mI) at 3.5–3.6 ppm. mI is a pentose sugar, which
is part of the inositol triphosphate intracellular second
messenger system. Levels have been found to be reduced in
hepatic encephalopathy, and increased in Alzheimer’s dementia
and demyelinating diseases. The exact pathophysiological
significance of alterations in mI is uncertain, but a leading
hypothesis is that elevated mI reflects increased populations of
glial cell, which are known to express higher levels of mI than
neurons; this may be related to differences in
myo-inositol/sodium co-transporter activity that appears to play
a key role in astrocyte osmoregulation. This would explain
chronic disturbance in mI both in degenerative and
inflammatory disease, and transiently in hypo- and hyperosmolar
states.
Myo-inositol resonates at almost the same frequency in the
spectrum as glycine; however, glycine is a singlet, while mI is
a strongly coupled multiplet, so the two can usually be
distinguished by using different echo times (glycine should be
the predominant signal at long echo times), or field strengths.
Glycine is usually at low concentration in the normal brain, but
can increase to detectable levels in some diseases, such as
nonketotic hyperglycinemia.
Glutamate and glutamine
Glutamate (Glu) and glutamine (Gln) are key compounds in brain
metabolism. Glutamate is the most abundant amino acid in the
brain, and is the dominant neurotransmitter. During neuronal
excitation, glutamate is released and diffuses across the
synapse, where it is rapidly taken up by astrocytes (along with
sodium ions (Na+)). The astrocyte converts the glutamate to
glutamine, which is then released and reuptaken by neurons. In
the neuron, glutamine is converted back to glutamate, and the
process repeated. This glutamate–glutamine cycling is an
energy-demanding process, which has been speculated to consume
as much as 80–90% of the total cortical glucose usage.
Since at a field strength of 1.5 T there is almost complete
overlap of Glu and Gln, they are usually labeled as a composite
peak Glx, and are very difficult to separate, although some
authors have attempted to distinguish them. The 2CH protons of
both Glu and Gln resonate around 3.7 ppm, while the 3CH2 and
4CH2 multiplets occur between 2.1 and 2.4 ppm. At 3 T, Glu and
Gln may be determined quite reliably with an appropriate pulse
sequence and/or curve fitting methods. At higher fields (at 4 T
or above), the 4CH2 resonances of Glu and Gln start to become
well resolved, and hence more reliably determined.
Because of the difficulty of measuring Glu and Gln at 1.5 T,
relatively few studies have looked at pathology-related changes
in these compounds. However, recently Glu was found to be
elevated in MS plaques at 3 T, and previous studies at 1.5 T
found elevated cerebral Gln in patients with liver failure (for
example, hepatic encephalopathy and Reye’s syndrome, most likely
as the result of increased blood ammonia levels, which increases
glutamine synthesis.
Less commonly detected compounds
A survey of the literature reveals more than 25 additional
compounds that have been assigned in proton spectra of the human
brain. Some of these compounds are present in the normal human
brain, but are difficult to detect routinely because they are
very small and/or have overlapping peaks. Some examples of these
compounds include NAAG, aspartate, taurine, scyllo-inositol,
betaine, ethanolamine, purine nucleotides, histidine, glucose
and glycogen. Other compounds are yet more difficult to detect,
and require the use of spectral editing pulse sequences in order
to be detected, because their resonances overlap almost
completely with those of other, more abundant, compounds.
Examples of these include γ-amino-butyric acid (GABA) and
glutathione.
Under disease conditions, certain compounds may become visible
as their concentration increases sufficiently high to be
detected. Examples of compounds that have been detected under
pathological conditions include the ketone bodies
β-hydroxybutyrate and acetone, and other compounds such as
phenylalanine (in phenylketonurea), galactitol, ribitol,
arabitol in polyol disease, and succinate, pyruvate, alanine,
glycine, and threonine in various disorders.
Exogenous compounds which are able
to cross the blood brain barrier may also reach sufficiently
high concentrations to be detected by proton MRS. Examples of
exogenous compounds, sometimes termed “xenobiotics”, include the
drug delivery vehicle propan-1,2-diol, mannitol (used to reduce
swelling and edema in neurosurgical procedures and intensive
care), ethanol, and the health food supplement
methyl-sulfonyl-methane (MSM).
In addition to metabolite concentrations, other information may
also be measured from brain proton spectra. For instance,
measurements of absolute (as
opposed to relative, as can be measured by MRI)
brain temperature have been made
using the water–NAA chemical shift difference (the water
chemical shift has a 0.01 ppm/°C temperature dependence, whereas
that of NAA is temperature-independent).
In addition, the exchangeable protons of metabolites resonating
downfield of water may be used to estimate
brain pH. These compounds (histidine, homocarnosine, and
the amide resonance of NAA) generally have low signal intensity,
but are detectable by the use of short echo times, appropriate
water suppression methods, and high magnetic field strengths.
Using oral loading of histidine (to increase its detectability),
Vermathen et al. were able to estimate brain pH from the
chemical shift difference of the C2 and C4 resonances of the
imidazole ring; similarly, Rothman et al. were able to use the
same resonances of homocarnosine to estimate brain pH in
epilepsy patients who were receiving therapy which caused
increased brain homocarnosine concentrations. The rate of
exchange of the NAA amide protons with water is also pH
sensitive, and can be used to estimate brain pH.
Compounds detected by proton MRS outside the CNS
The discussion so far has focused entirely on the information
content of proton spectra of the human brain; however, when
going to other organ systems, different compounds are detected
in the spectra – for instance, in normal prostate tissue, a
signal from citrate at 2.6 ppm is typically detected, while
normal breast tissue usually only contains visible water and fat
signals. In muscle, signals may be detected from intra- and
extramyocellular lipids, acetylcarnotine, creatines, cholines,
taurine and carnosine.
 |
Sequences |
 |
(MRS / MRSI - Magnetic
Resonance Spectroscopic Imaging) A method using
the NMR phenomenon to identify the chemical
state of various elements without destroying the
sample. MRS therefore provides information about
the chemical composition of the tissues and the
changes in chemical composition, which may occur
with disease processes.
Although MRS is primarily employed as a research
tool and has yet to achieve widespread
acceptance in routine clinical practice, there
is a growing realization that a noninvasive
technique, which monitors disease biochemistry
can provide important new information for the
clinician.
The underlying principle of MRS is that atomic
nuclei are surrounded by a cloud of electrons,
which very slightly shield the nucleus from any
external magnetic field. As the structure of the
electron cloud is specific to an individual
molecule or compound, then the magnitude of this
screening effect is also a characteristic of the
chemical environment of individual nuclei.
In view of the fact that the resonant frequency
is proportional to the magnetic field that it
experiences, it follows that the resonant
frequency will be determined not only by the
external applied field, but also by the small
field shift generated by the electron cloud.
This shift in frequency is called the chemical
shift (see also Chemical Shift). It should be
noted that chemical shift is a very small
effect, usually expressed in ppm of the main
frequency. In order to resolve the different
chemical species, it is therefore necessary to
achieve very high levels of homogeneity of the
main magnetic field B0. Spectra from humans
usually require shimming the magnet to
approximately one part in 100. High resolution
spectra of liquid samples demand a homogeneity
of about one part in 1000.
In addition to the effects of factors such as
relaxation times that can affect the NMR signal,
as seen in magnetic resonance imaging, effects
such as J-modulation or the transfer of
magnetization after selective excitation of
particular spectral lines can affect the
relative strengths of spectral lines.
In the context of human MRS, two nuclei are of
particular interest - H-1 and P-31. (PMRS -
Proton Magnetic Resonance Spectroscopy) PMRS is
mainly employed in studies of the brain where
prominent peaks arise from NAA, choline
containing compounds, creatine and creatine
phosphate, myo-inositol and, if present,
lactate; phosphorus 31 MR spectroscopy detects
compounds involved in energy metabolism
(creatine phosphate, adenosine triphosphate and
inorganic phosphate) and certain compounds
related to membrane synthesis and degradation.
The frequencies of certain lines may also be
affected by factors such as the local pH. It is
also possible to determine intracellular pH
because the inorganic phosphate peak position is
pH sensitive.
If the field is uniform over the volume of the
sample, "similar" nuclei will contribute a
particular frequency component to the detected
response signal irrespective of their individual
positions in the sample. Since nuclei of
different elements resonate at different
frequencies, each element in the sample
contributes a different frequency component. A
chemical analysis can then be conducted by
analyzing the MR response signal into its
frequency components.
Binomial Pulses
A sequence of two or more pulses with a null response at a
particular frequency used to suppress the water signal in
localized proton spectroscopy.
Chemical Shift Imaging
(CSI) Chemical shift imaging is an extension of MR spectroscopy,
allowing metabolite information to be measured in an extended
region and to add the chemical analysis of body tissues to the
potential clinical utility of Magnetic Resonance. The spatial
location is phase encoded and a spectrum is recorded at each
phase encoding step to allow the spectra acquisition in a number
of volumes covering the whole sample. CSI provides mapping of
chemical shifts, analog to individual spectral lines or groups
of lines.
Spatial resolution can be in one, two or three dimensions, but
with long acquisition times od full 3D CSI. Commonly a
slice-selected 2D acquisition is used. The chemical composition
of each voxel is represented by spectra, or as an image in which
the signal intensity depends on the concentration of an
individual metabolite. Alternatively frequency-selective pulses
exite only a single spectral component.
There are several methods of performing chemical shift imaging,
e.g. the inversion recovery method, chemical shift selective
imaging sequence, chemical shift insensitive slice selective RF
pulse, the saturation method, spatial and chemical shift encoded
excitation and quantitative chemical shift imaging.
Chemical Shift Selective Imaging Sequence
(CHESS) A sequence for water suppression in proton MR
spectroscopy and for water or fat suppression in MR imaging.
This technique uses a frequency-selective 90° pulse to
selectively excite the water signal, followed by a spoiler
gradient to dephase the resulting magnetization. The gradients
may be repeated several times in different directions to
increase its effectiveness.
Depth Resolved Spectroscopy
(DRESS) Depth resolved surface spectroscopy is a localization
method that employ gradients to select the region from which
spectra are acquired.
Point Resolved Spectroscopy
(PRESS) Point resolved spectroscopy is a multi echo single shot
technique to obtain spectral data. PRESS is a 90°-180°-180°
(slice selective pulses) sequence. The 90° radio frequency pulse
rotates the spins in the yx-plane, followed by the first 180°
pulse (spin rotation in the xz-plane) and the second 180° pulse
(spin rotation in the xy-plane), which gives the signal.
With the long echo times used in PRESS, there is a better
visualization of metabolites with longer relaxation times. Many
of the metabolites depicted by stimulated echo technique are not
seen on point resolved spectroscopy, but PRESS is less
susceptible to motion, diffusion, and quantum effects and has a
better SNR than stimulated echo acquisition mode (STEAM).
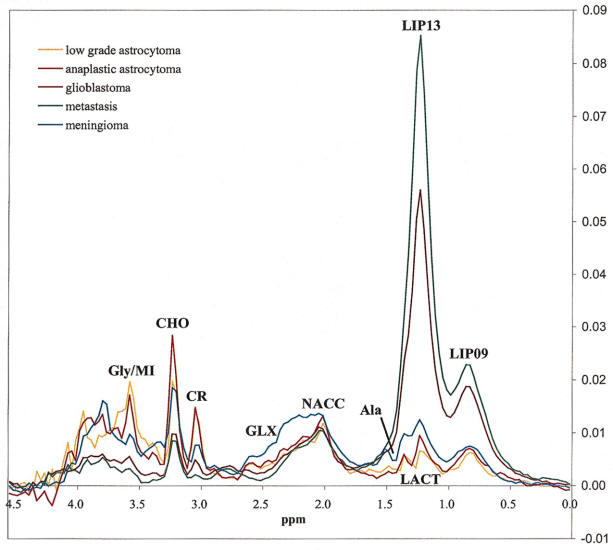 |
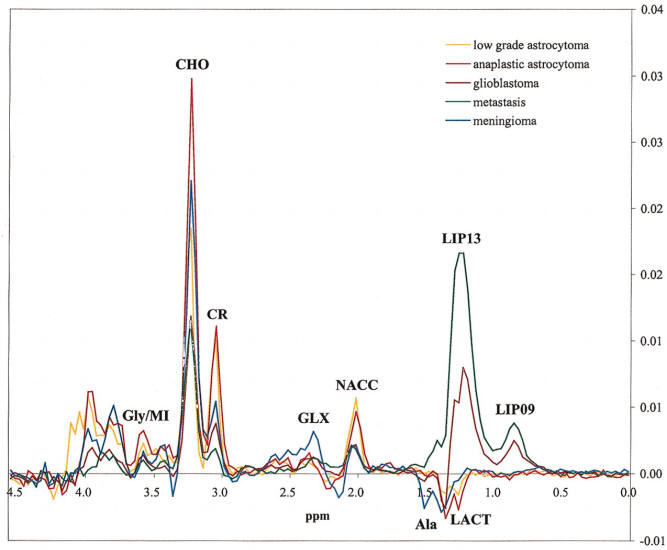 |
Long TE 136 msec spectroscopy |
Short TE 30 msec spectroscopy |
Spectroscopy Evaluation
Integrated software package with extensive graphical display
functionality to evaluate and post-process spectroscopy
acquisition data.
Features
Display of CSI data as colored metabolite images or spectral
overview maps, overlaid on anatomical image
Export of spectroscopy data to a user-accessible file format
Relative quantification of spectra, compilation of the data to
result table
Automated peak normalization tissue, water or reference
New dedicated SVS breast evaluation protocols
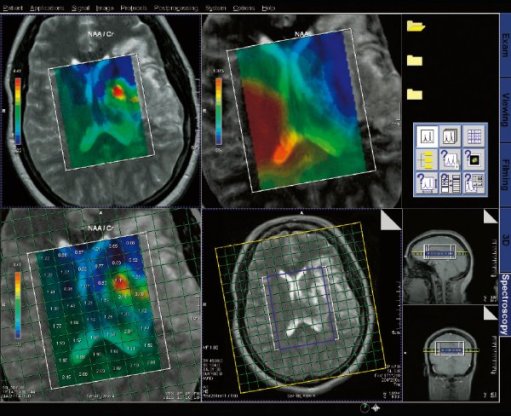 |
Spectroscopy evaluation task card |
Step by step for basic functionality (SVS)
1. Load the SVS data set into the Spectroscopy application.
The metabolite spectrum will automatically be shown in the first
segment.
2. Select single data set mode. This will allow for creation of
tables in the empty segments.
3. View the localizer. Double clicking on a localizer image puts
this image in the large segment.
4. Activate an empty segment and right click select results
table. This table will give you a ratio of metabolite integrals,
where you select the denominator.
5. Save the results. Activate a segment and select save as,
choose "selected results". This can be viewed or sent to PACs
from the patient browser.
Step by step for basic functionality (CSI) 1. Load
the CSI data set into the Spectroscopy Application.
2. Select Spectral Map on an empty segment. This will provide
spectral graphs for all voxel within the Vol.
3. Zoom and pan the image to the desired size.
4. Select the last empty segment and select metabolite map.
Create a ratio map to show levels of a desired metabolite
compared to another.
5. Zoom and pan this image to the desired size.
6. Select the Save Data icon, Selected Results, to save maps.
7. Individual voxel graphs can be viewed by selecting a voxel on
the localizer
Single Voxel Spectroscopy
Software package with sequences and protocols
for single voxel proton spectroscopy.
Features
Streamlined for easy push-button operation
Matrix Spectroscopy – phase-coherent signal
combination from several coil elements for
maximum SNR based on the head matrix coil
Spectral suppression (user definable parameter)
to avoid lipid superposition in order to
reliably detect e.g. choline in the breast
Up to 8 regional saturation (RSat) bands for
outer volume suppression can be defined by the
user
Physiological triggering (ECG, pulse,
respiratory or external trigger) in order to
avoid e.g. CSF pulsation artifacts
|
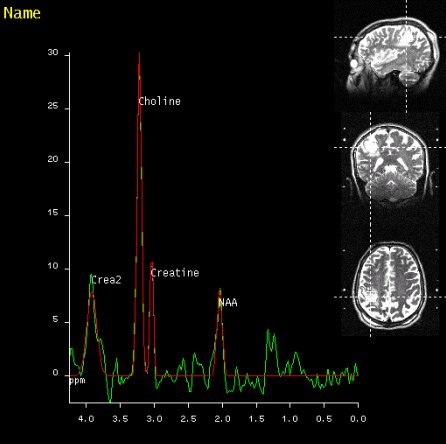 |
|
SVS shows increased Choline signal
in the lesion of the right parietal
lobe, proving malignancy |
Step by step:
1. Run localizers, and open the svs_se_135
sequence. This is found in the exam explorer,
under; Spectroscopy, Head, SVS.
2. Position the VOI on one image and go to
scroll, nearest. This will align the VOI in
plane for all orientations.
3. Notice that the VOI has solid borders. This
means that the VOI intersects in all three
planes.
4. Select "Reference Lines". This will show
where the slices intersect the VOI.
5. Apply the sequence.
6. Notice in this example that the sequence name
has been changed from the factory default
nomenclature.
This will disable the automatic postprocessing
protocol when loaded into Spectroscopy
Evaluation. To correct this ensure the scanned
sequence stays the same as the factory default.
GRACE: GeneRAlized breast speCtroscopy Exam- Choline
level follow up to evaluate Ca breast: (GeneRAlized breast
speCtroscopy Exam) SVS technique (spin echo sequence) optimized for breast
spectroscopy. The technique contains a special spectral lipid suppression
pulse (user definable) for lipid signal reduction. Siemens
unique water reference detection to visualize the normalized
choline ratio. Online frequency shift correction for reduction of breathing
related artifacts, Inline implementation – no additional
user interaction is required. Clinical applications: • Differentiating benign from malignant breast lesions • Predicting clinical response to neoadjuvant chemotherapy
in an early stage (24hours after receiving the first dose)
|
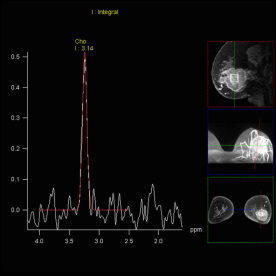 |
3D CSI (Chemical Shift Imaging): Integrated multivoxel spectroscopy software package with
sequences and protocols for 3D Chemical Shift Imaging (CSI). Features Matrix Spectroscopy – phase-coherent signal combination from
several coil elements for maximum SNR with configurable
prescan-based normalization for optimal homogeneity 3D Chemical Shift Imaging Hybrid CSI with combined Volume selection and Field of View
(FoV) encoding Short TEs available (30 ms for SE, 20 ms for STEAM) Automized shimming of the higher order shimming channels for
optimal homogeneity of the larger CSI volumes Weighted acquisition, leading to a reduced examination time
compared to full k-space coverage while keeping SNR and
spatial resolution Outer Volume Suppression Spectral Suppression Protocols for prostate spectroscopy Clinical Applications Prostate Spectroscopy for diagnosis, localization of
prostate cancer Improved spatial localization of metabolic changes in biopsy
or radiotherapy planning
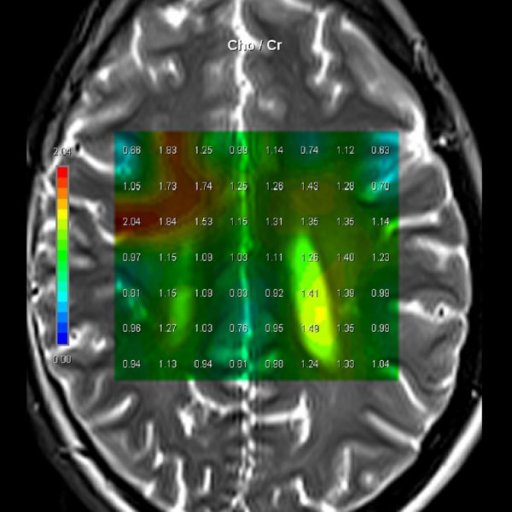 |
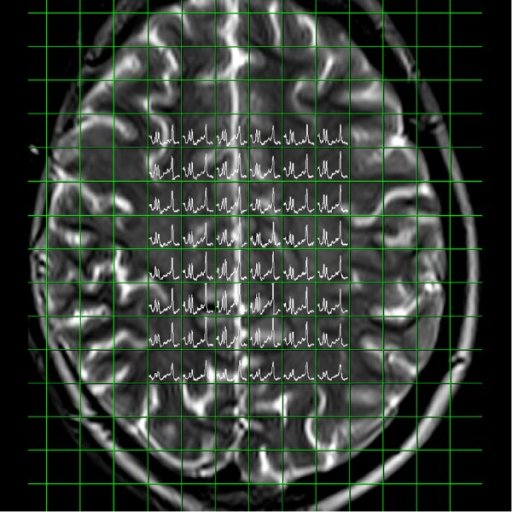 |
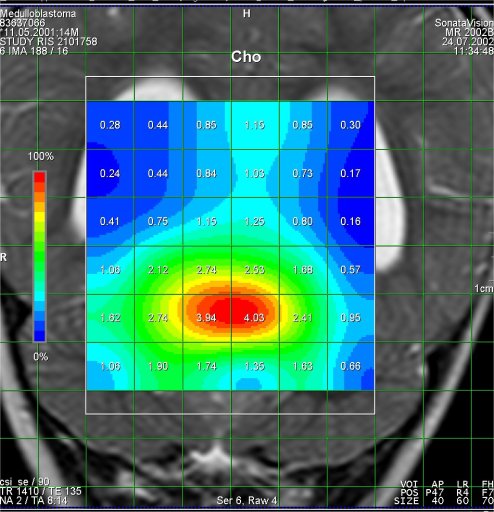 |
Cho/Cr ratio map
generated from 3D CSI measurement |
Spectral nap
generated from 3D CSI measurement |
Increased Cho-signal
in a medulloblastoma case |
Step by step:
1. Perform imaging in all three planes to
include the entire brain. Open the csi 3D se 135
sequence. Located in the exam explorer in the
Spectroscopy, CSI, head region.
2. Scroll thru the transversal images for area
of interest.
3. Copy image position. Right click on the
selected transverse image, from the menu select
copy image position.
4. Go to the scroll drop down menu and select
scroll nearest. This will align the 3D VOI in
all three orientations.
5. Rotate the VOI inplane on the transversal
image to cover the area of interest.
6. Open toolbar, and and select create sat
bands. Draw saturation bands around all sides of
the 3D VOI to remove lipid signal from
calvarium.
7. Select fully excited VOI, on the Geometry
card.
Apply the sequence.
31 P Spectroscopy: Optimized for liver and heart
applications.
Integrated package with RF coil, sequences and protocols for
31P spectroscopy.
Offering the same level of user friendliness and automation
as 1H spectroscopy.
1H/31P transmit/ receive Heart Liver coil for 31P
spectroscopy
Short TE CSI sequence and protocols optimized for heart and
liver applications
NOE (Nuclear Overhauser Effect) and 1H decoupling available
ECG triggering available
Weighted acquisition available
 |
Prostate
Package #T+D |
 |
The prostate spectroscopy package is an comprehensive
software package which bundles:
- Single Voxel Spectroscopy
- 2D Chemical shift Imaging
- 3D Chemical Shift Imaging
- Spectroscopy Evaluation syngo
- syngo Tissue 4D Evaluation
Sequences and protocols for proton spectroscopy, 2D and 3D
proton chemical shift imaging (2D CSI and 3D CSI) to examine
metabolic changes in the prostate are included. Furthermore
included is the comprehensive Spectroscopy evaluation software
which enables fast evaluation of spectroscopy data on the syngo
Acquisition Workplace.
Tissue 4D is an application for visualizing and post-processing
dynamic contrast-enhanced 3D datasets.
Tissue 4D provides two evaluation options:
- Standard curve evaluation
- Curve evaluation according to a pharmacokinetic model.
The spectroscopy evaluation software is fully integrated in
syngo MR.
Evaluation protocols adapted to the scan protocols carry out a
complete and automatic evaluation of the measured data.
Optimized protocols for 3D CSI in the prostate are included.
The following functions are included:
- Subsequent water suppression with optional phase correction
- Apodization
- Zero filling
- Fourier transformation
- Base line correction
- Automatic or manual phase correction
- Curve fitting and peak labeling
- Summaries in tabular form of the essential results specifying
the metabolites, their position, integrals and signal ratios in
relation to a selectable reference.
Tissue 4D provides the tissue visualization features:
- 4D visualization (3D and over time)
- Color display of parametric cards (Ktrans, Kep, Ve, Vp, iAUC)
- Additional visualization of 2D or 3D morphological dataset
Post-processing features:
- Elastic 3D motion correction
- Fully automatic calculation of subtracted images
Standard curve evaluation:
- Calculation and display of enrichment curves
Pharmacokinetic model:
- Pharmacokinetic calculation on a pixel-by-pixel basis using a
2-compartment model
- Calculation is based on the Toft model. Various model
functions are available.
- Manual segmentation and calculation on the result images.
The following result images can be saved as DICOM images:
- 3D motion-corrected, dynamic images
- Colored images
- Possibility for exporting results in the relevant layout
format.
 |
Applications
of Spectroscopy |
 |
In (1H) Magnetic Resonance Spectroscopy each proton can be
visualized at a specific chemical shift (peak position along
x-axis) depending on its chemical environment. This chemical
shift is dictated by neighboring protons within the molecule.
Therefore, metabolites can be characterized by their unique set
of 1H chemical shifts. The metabolites that MRS probes for have
known (1H) chemical shifts that have previously been identified
in NMR spectra. These metabolites include:
1.) N-acetyl Aspartate (NAA): with its major resonance peak at
2.02ppm, is a neuronal marker and decrease in levels of NAA indicate loss or damage to
neuronal tissue, which results from many types of insults to the
brain. Its presence in normal conditions indicates neuronal and
axonal integrity.
2.) Choline: with its major peak at 3.2ppm, choline is known to
be associated with membrane turnover, or increase in cell
division. Increased choline indicates increase in cell
production or membrane breakdown, which can suggest
demyelination or presence of malignant tumors or inflammatory
processes.
3.) Creatine & phosphocreatine: with its major peak at 3.0ppm,
creatine marks metabolism of brain energy. Gradual loss of
creatine in conjunction with other major metabolites indicates
tissue death or major cell death resulting from disease, injury
or lack of blood supply. Increase in creatine concentration
could be a response to cerebral trauma. Absence of creatine may
be indicative of a rare congenital disease.
4.) Lipids: with their major aliphatic peaks located in the
0.9-1.5ppm range, increase in lipids is seen is also indicative
of necrosis. These spectra are easily contaminated, as lipids
are not only present in the brain, but also in other biological
tissue such as the fat in the scalp and area between the scalp
and skull.
5.) Lactate: is a market of oxygen deficiency, reveals itself as a doublet (two symmetric peaks in
one) at 1.33ppm. Normally lactate is not visible, for its
concentration is lower that the detection limit of MRS, however
presence of this peak indicates glycolysis has been initiated in
an oxygen deficient environment. Several causes of this include
ischemia, hypoxia, mitochondrial disorders, and some types of
tumors.
6.) Myo-inositol: with its major peak at 3.56ppm, an increase in
Myo-inositol has been seen in granulation and gliosis and patients with Alzheimer’s,
dementia, and HIV patients.
7.) Glutamate and Glutamine: these amino acids are marked by a
series of resonance peaks between 2.2 and 2.4ppm.
Hyperammonemia, hepatic encephalopathy are two major conditions
that result in elevated levels of glutamine and glutamate. MRS,
used in conjunction with MRI or some other imaging technique,
can be used to detect changes in the concentrations of these
metabolites, or significantly abnormal concentrations of these
metabolites.
 |
Indication for Spectroscopy |
 |
Differential diagnosis of low-grade and high=grade tumors.
Monitoring under radio-chemotherapy.
differentiation of recurrent tumor from secondary necrosis due
to therapy.
 |
Spectroscopy
In Case of PNET |
 |
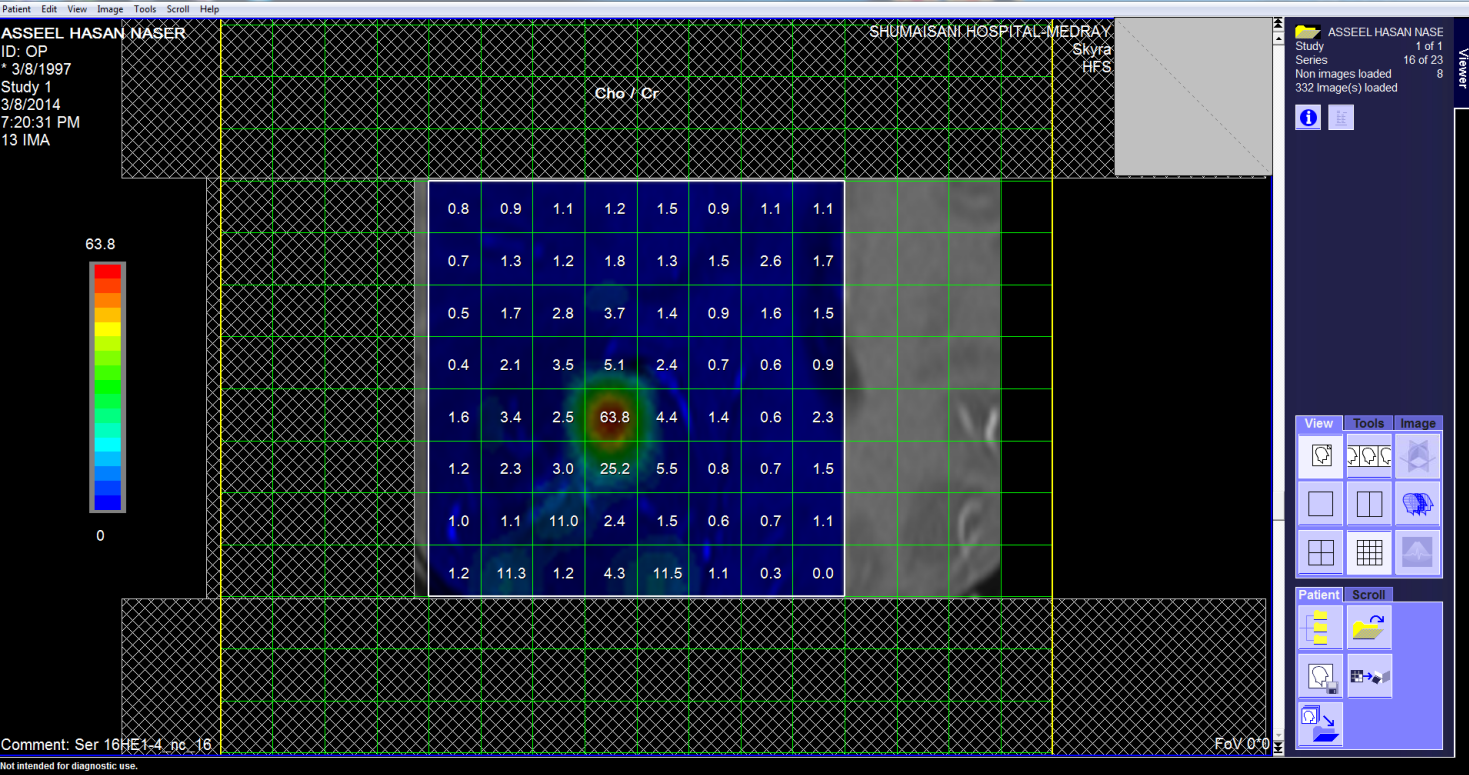
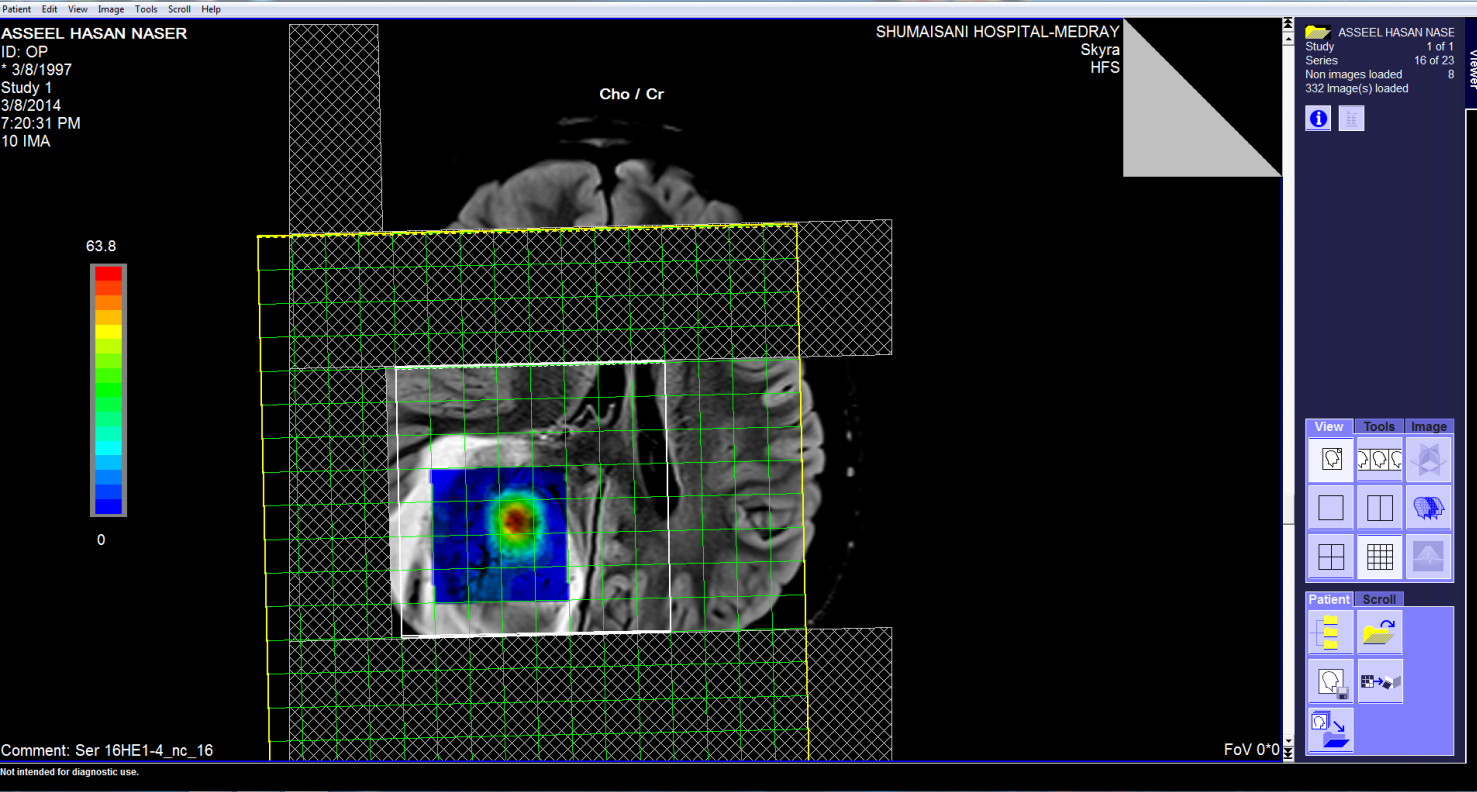
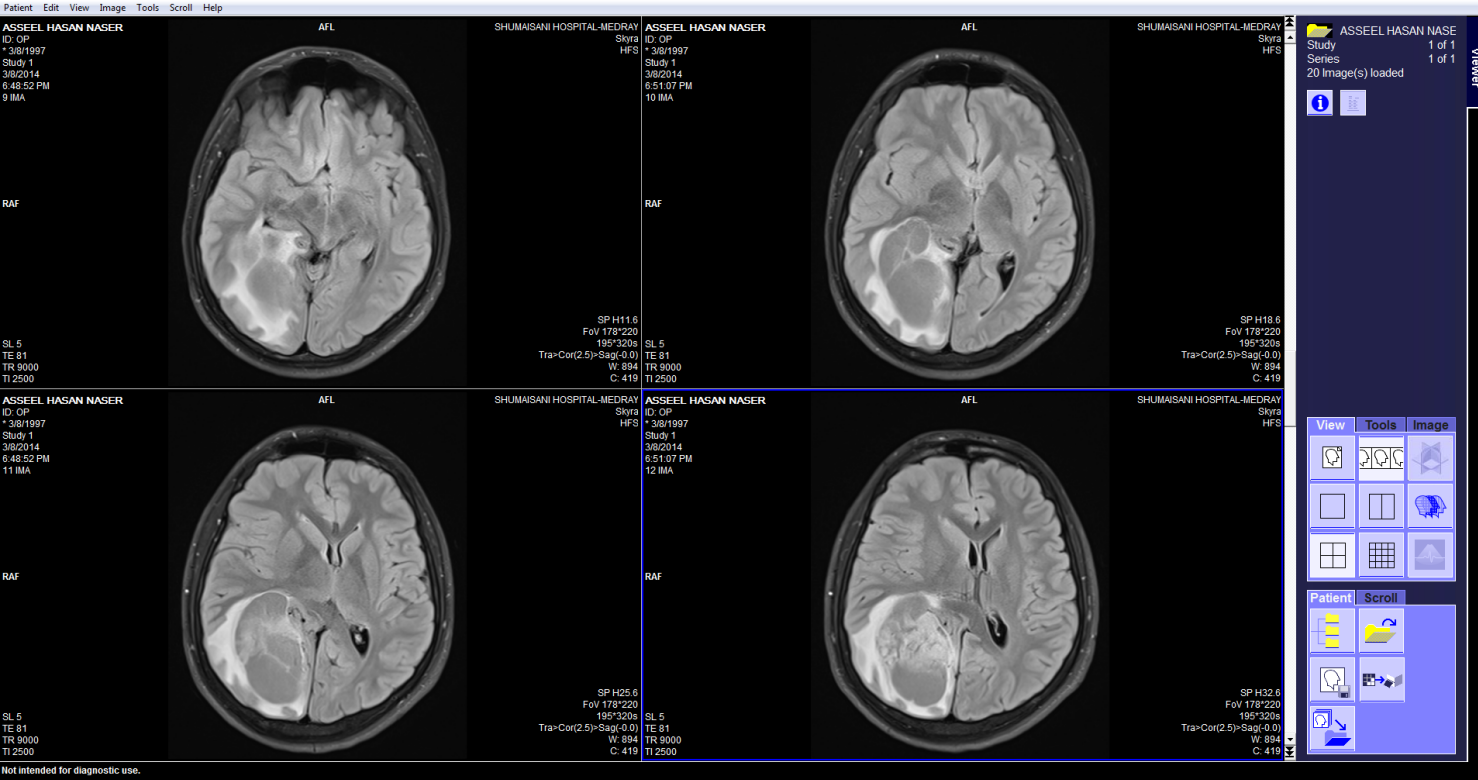
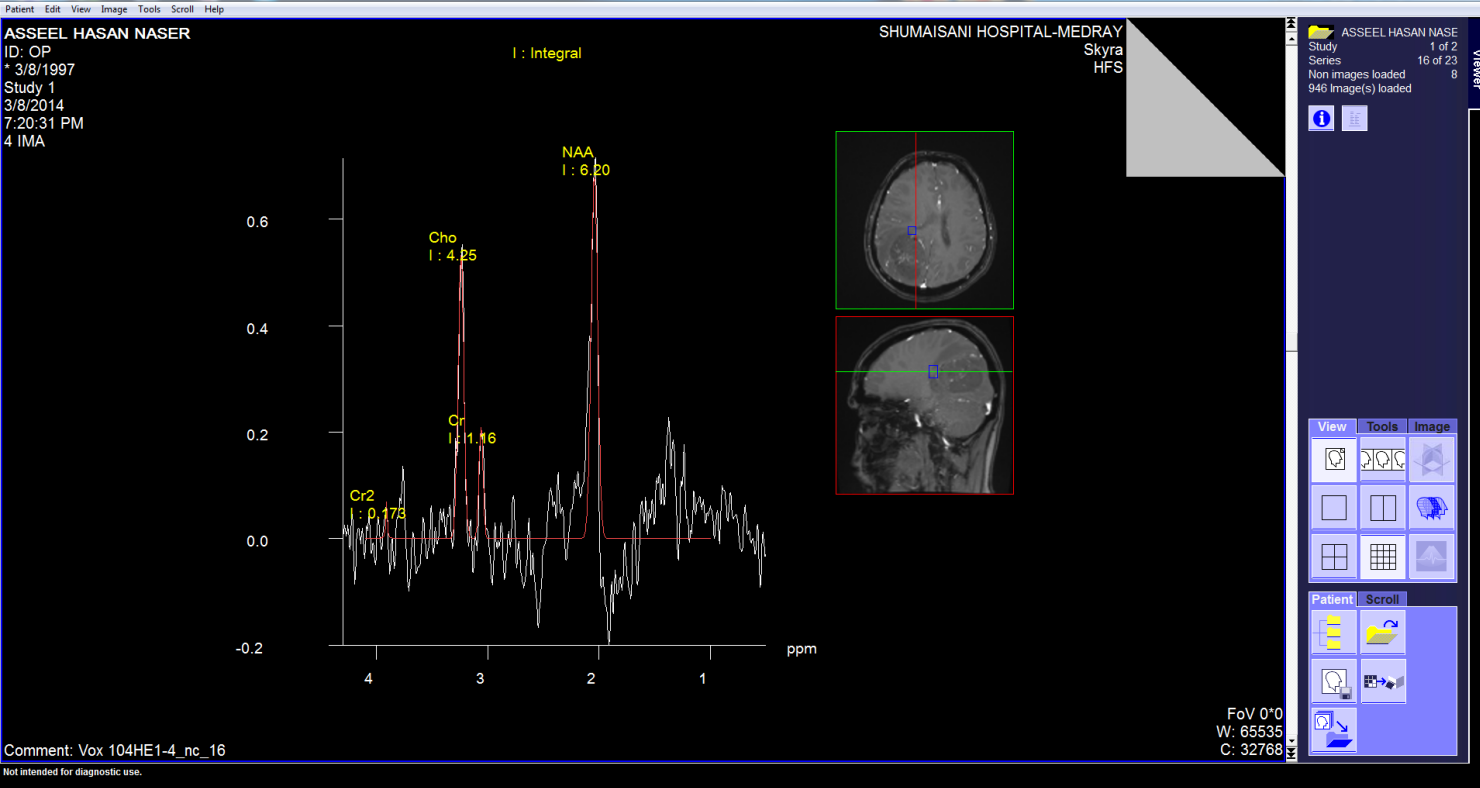
These pictures showing the case of 17 years age boy with highly
malignant tumor PNET (Primitive neuroectodermal tumor). Notice
the very malignant area where the Ch:Cr ratio reaching 63:1 and
the rapid slope at the nearby boundaries and the relatively high
NAA, indicating the presence of rapid cell proliferation.
 |
Spectroscopy
In Case of Pilocytic astrocytoma |
 |
The patient came to the clinic 03-June-2014 with the family,
telling that the patient the last month got headache with
vomiting and fainting attacks, ataxia and blurred vision.
CT-scan done today showing a mass with the diagnosis of
medulloblastoma.
On examination at the patient has no meningism, nor nystagmus.
The patient except for the above mentioned complains was
neurologically free.
The patient was sent for MR investigations with MRS and DTI. The
conventional MRI data and the spectroscopy were in favor of
policytic astrocytoma.
In setting position, midline posterior approach with reflection
of the bone flap to the neck was done. The dura was opened to
expose both cerebellar hemisphere more to the left. The nidus of
the tumor was fleshy violate and it was possible to resect all
of it and sent for fresh frozen sections which confirmed the
presence of pilocytic astrocytoma. Total resection of the cystic
mass with its fleshy contents was achieved. The vermian and the
tonsilar parts of the tumor were also included in the resection.
After strict hemostasis, the wound was closed temporarily and
the patient sent to the MRI. There is air over the left cerebral
hemisphere and a suspected remnant near the removed left tonsil.
The patient sent back to the operating table and in supine
position, the surgery was continued. The suspected mass in the
new MRI data was resected. Routine closure of the wound.
Smooth postoperative recovery. The patient sent to ICU for
observation.
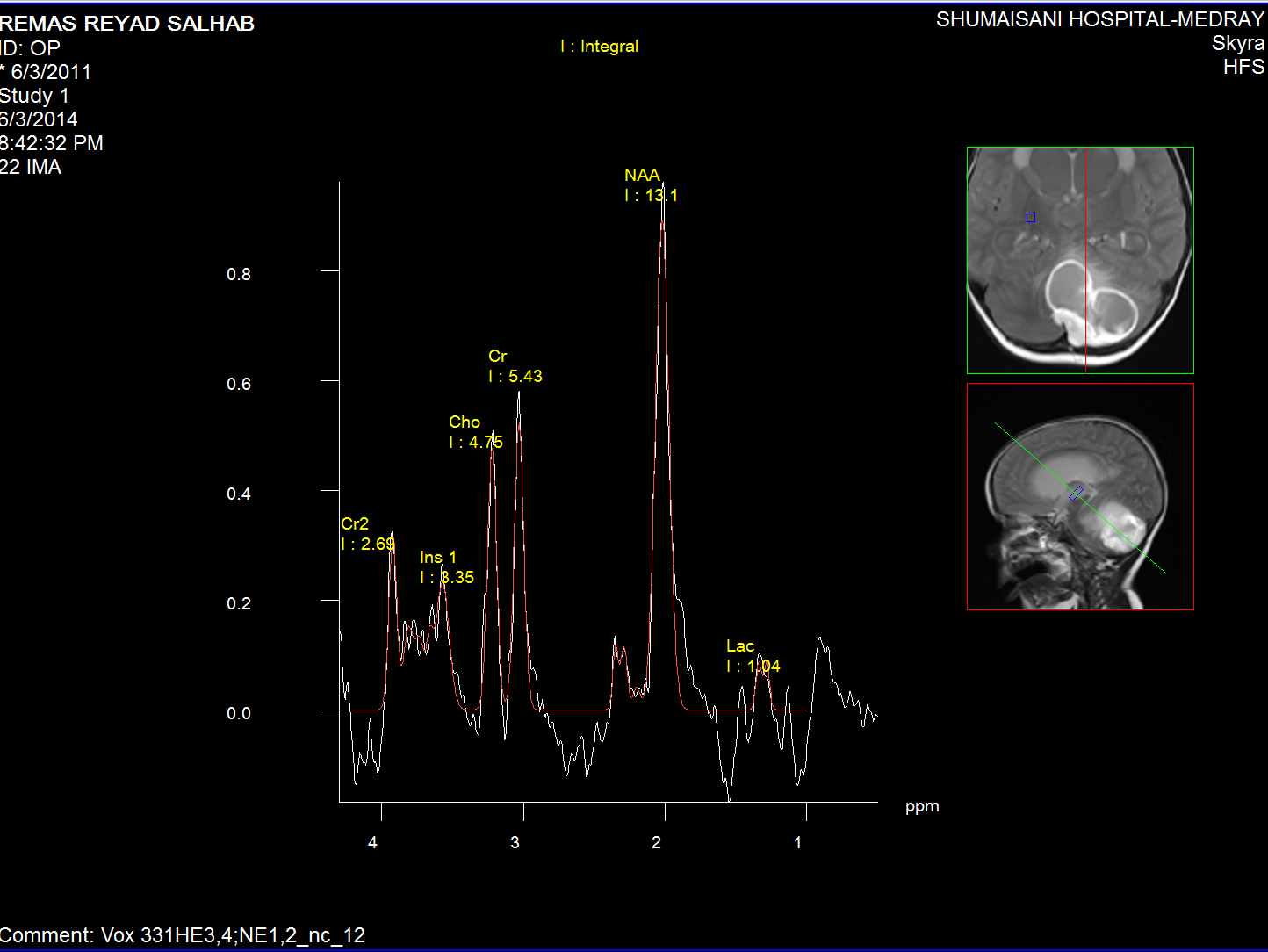
Normal brain MR Spectroscopy.
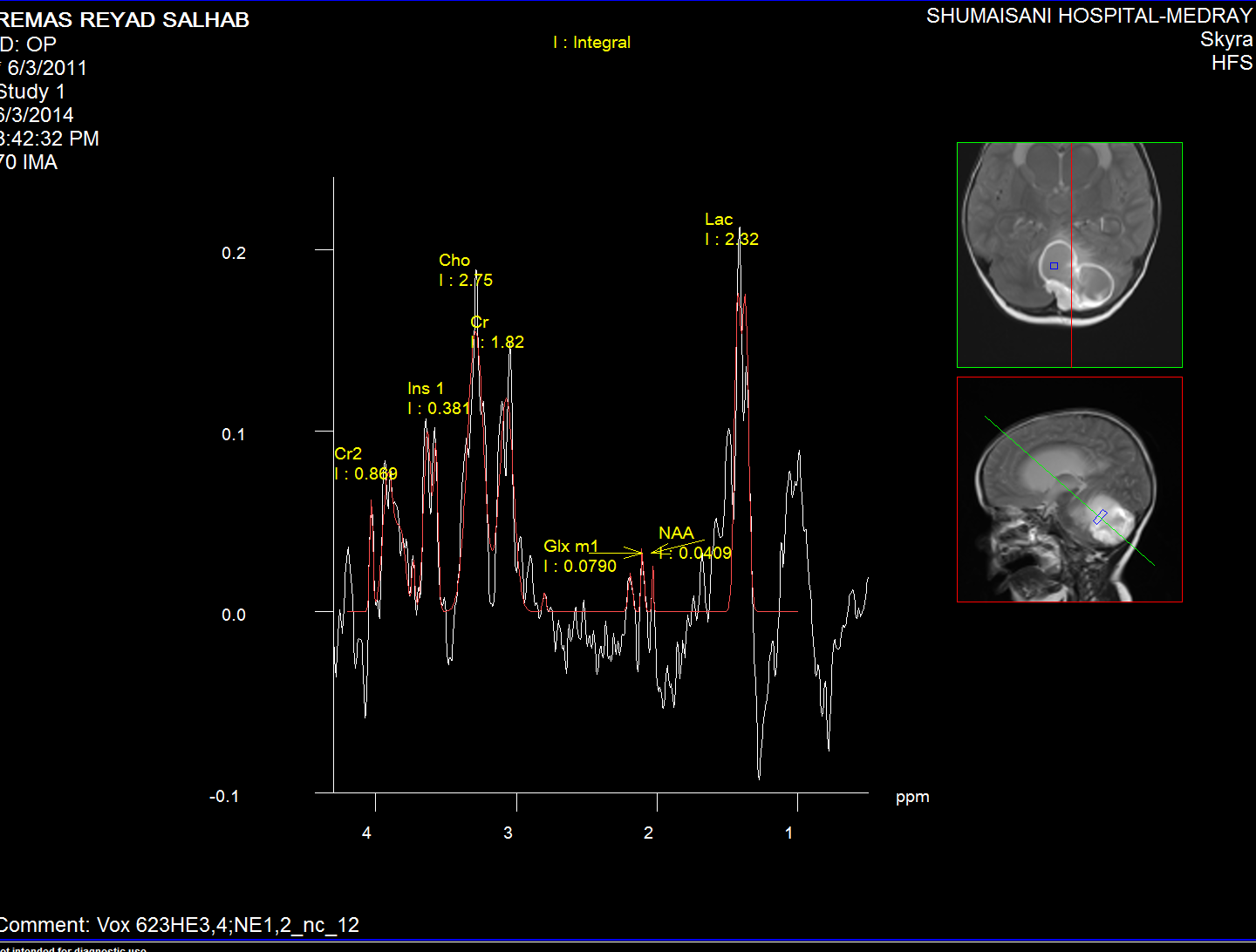
Spectroscopy of the intracyst material
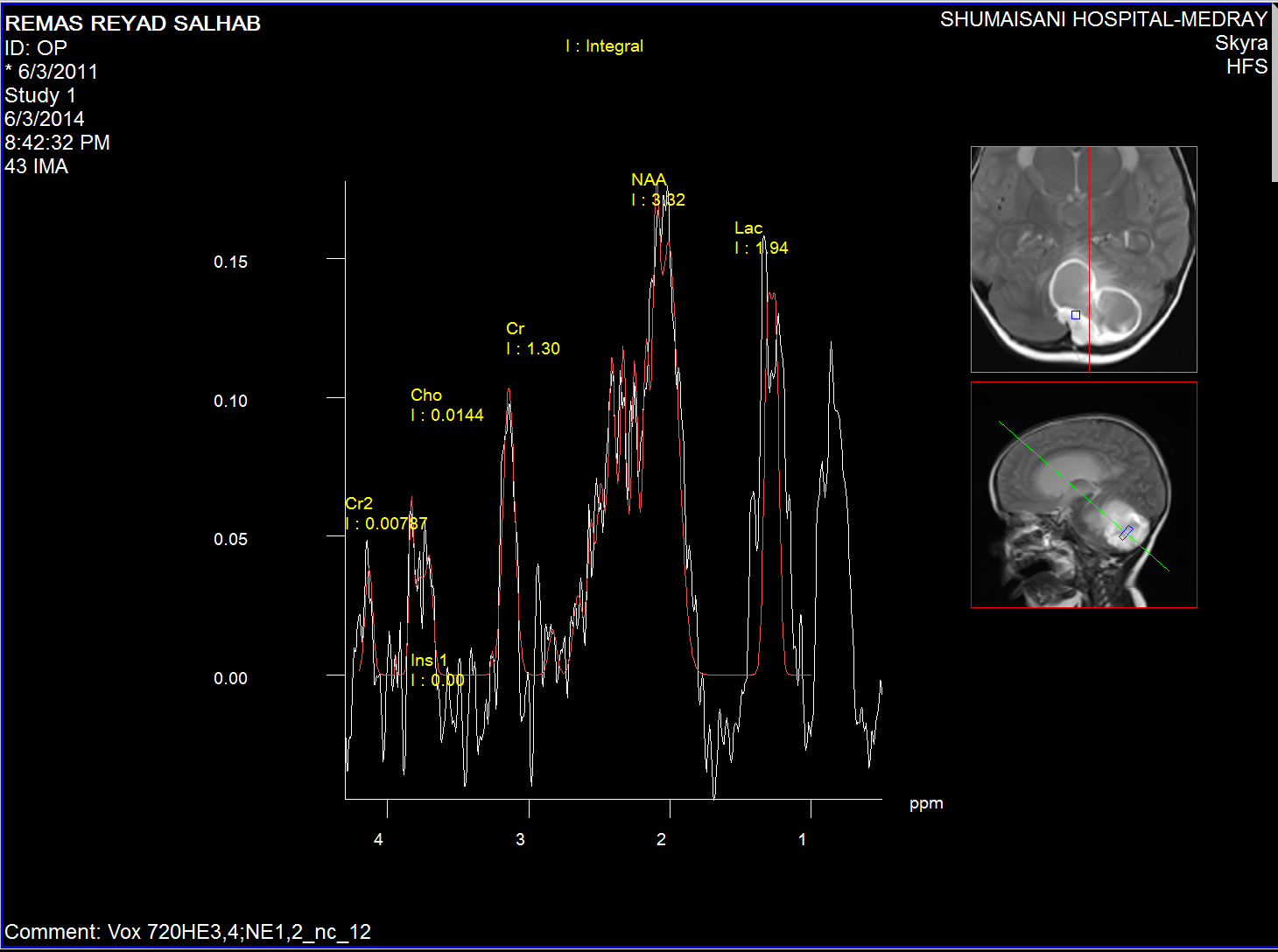
Spectroscopy of the nidus material.
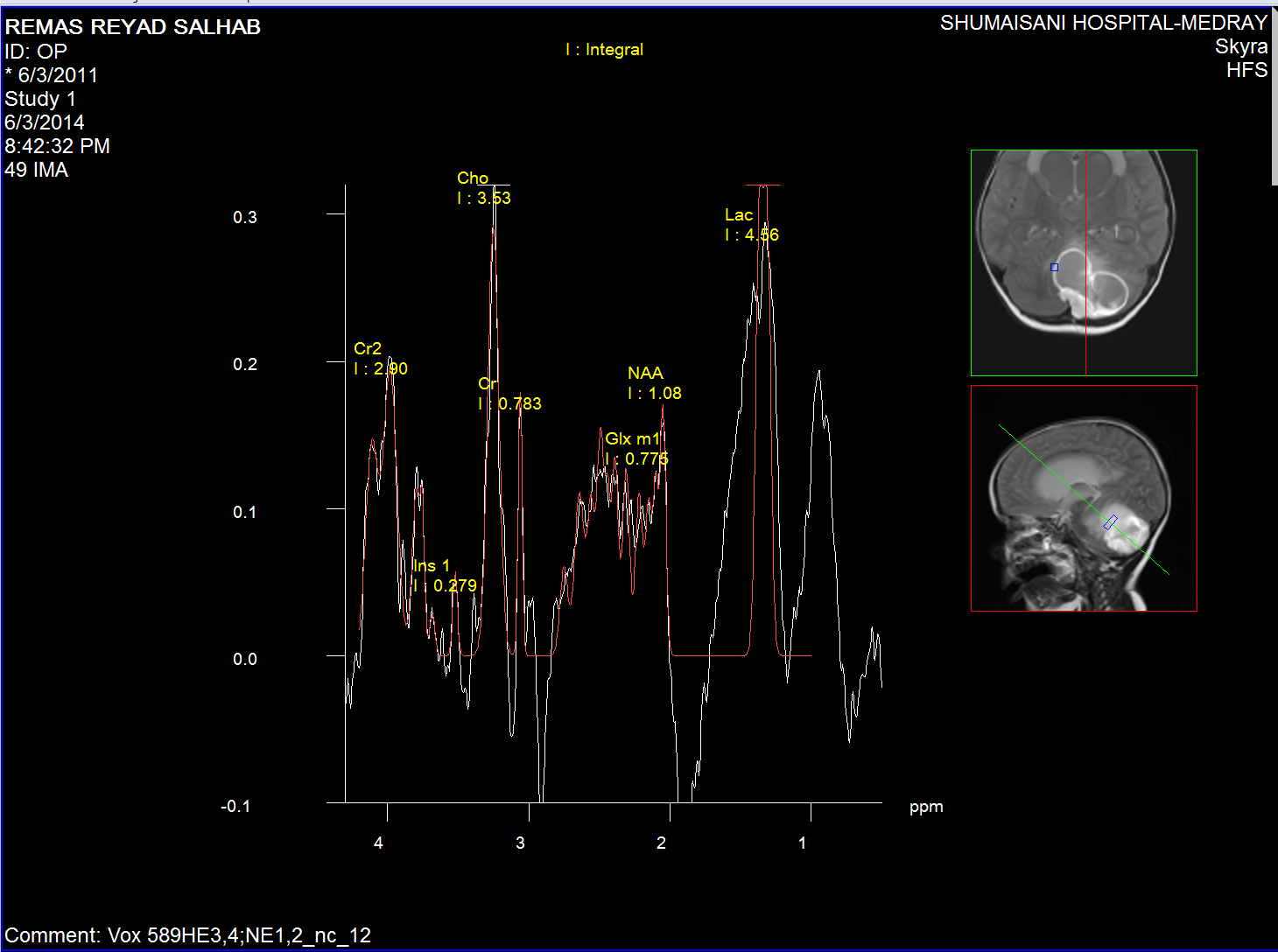
Cystic wall spectroscopy
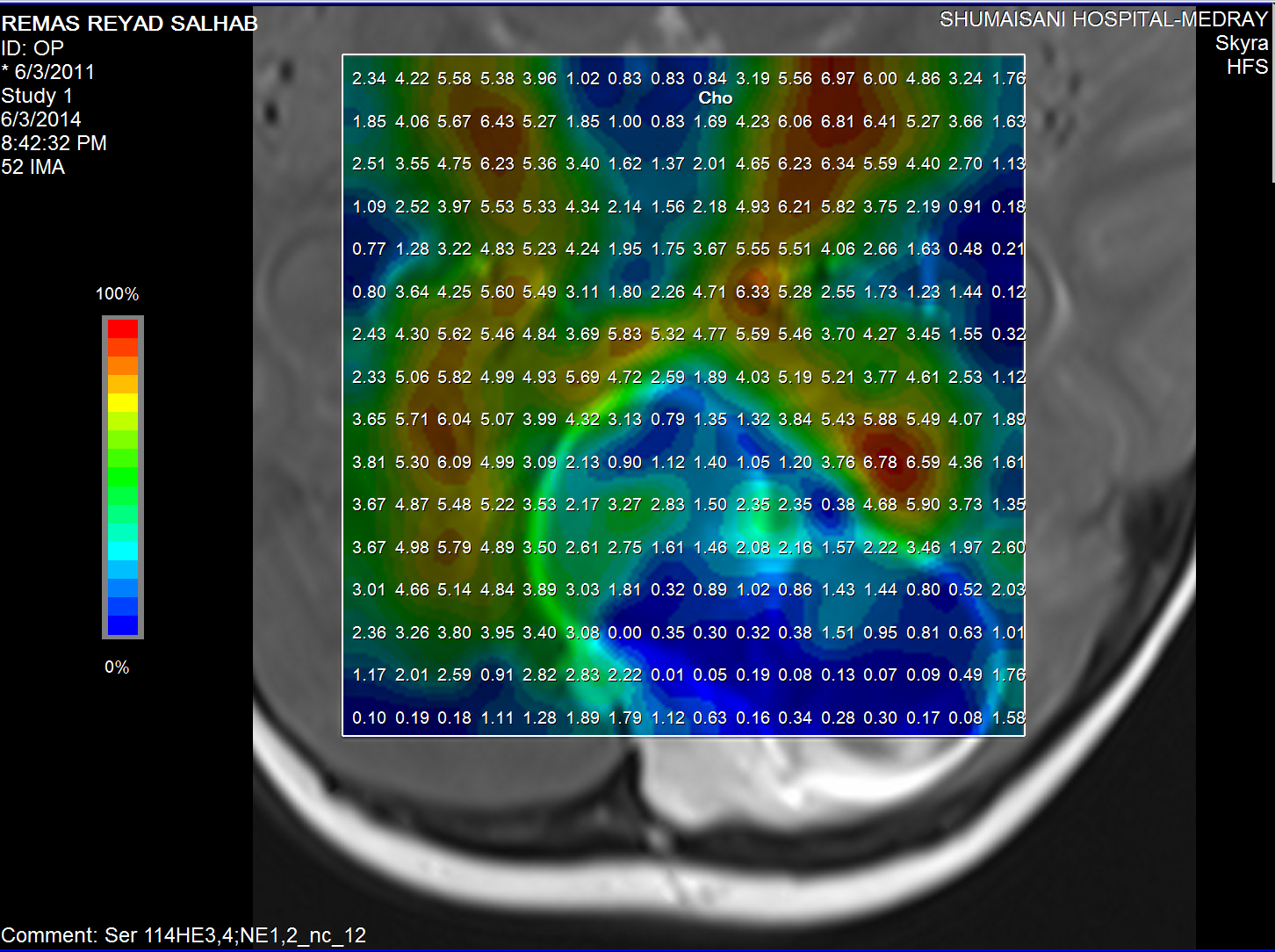
Choline distribution.
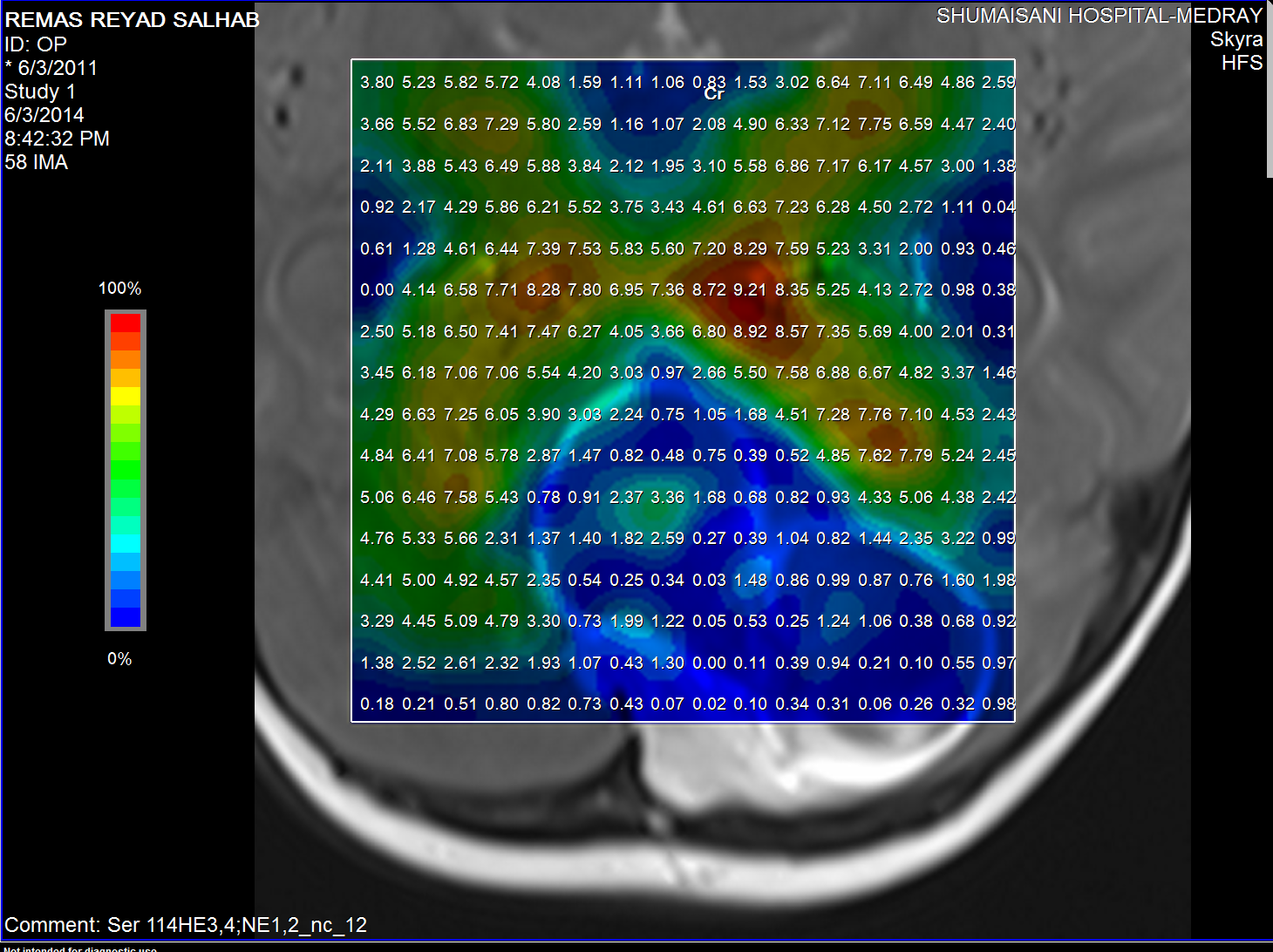
Creatine distribution
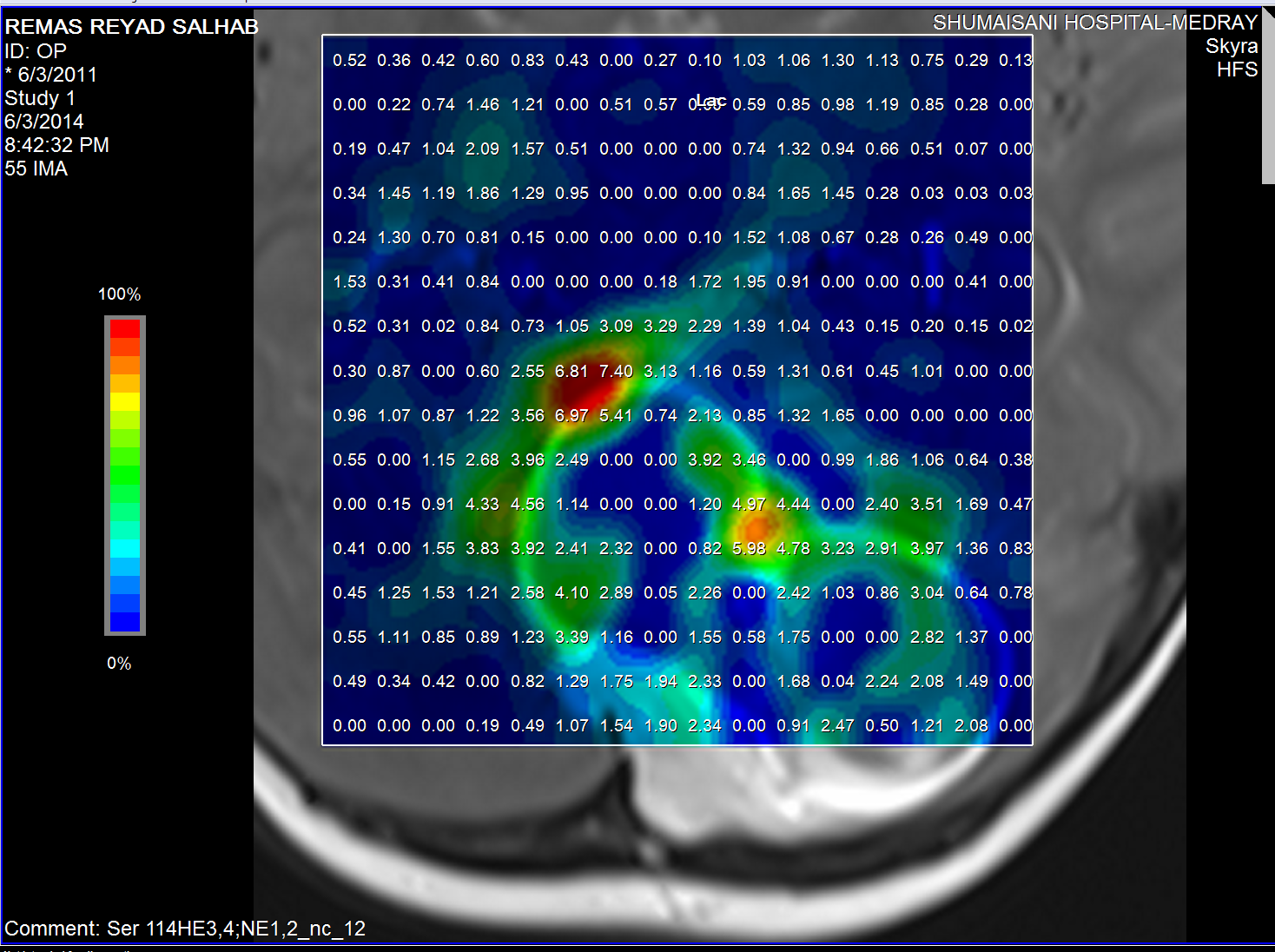
Lactate distribution
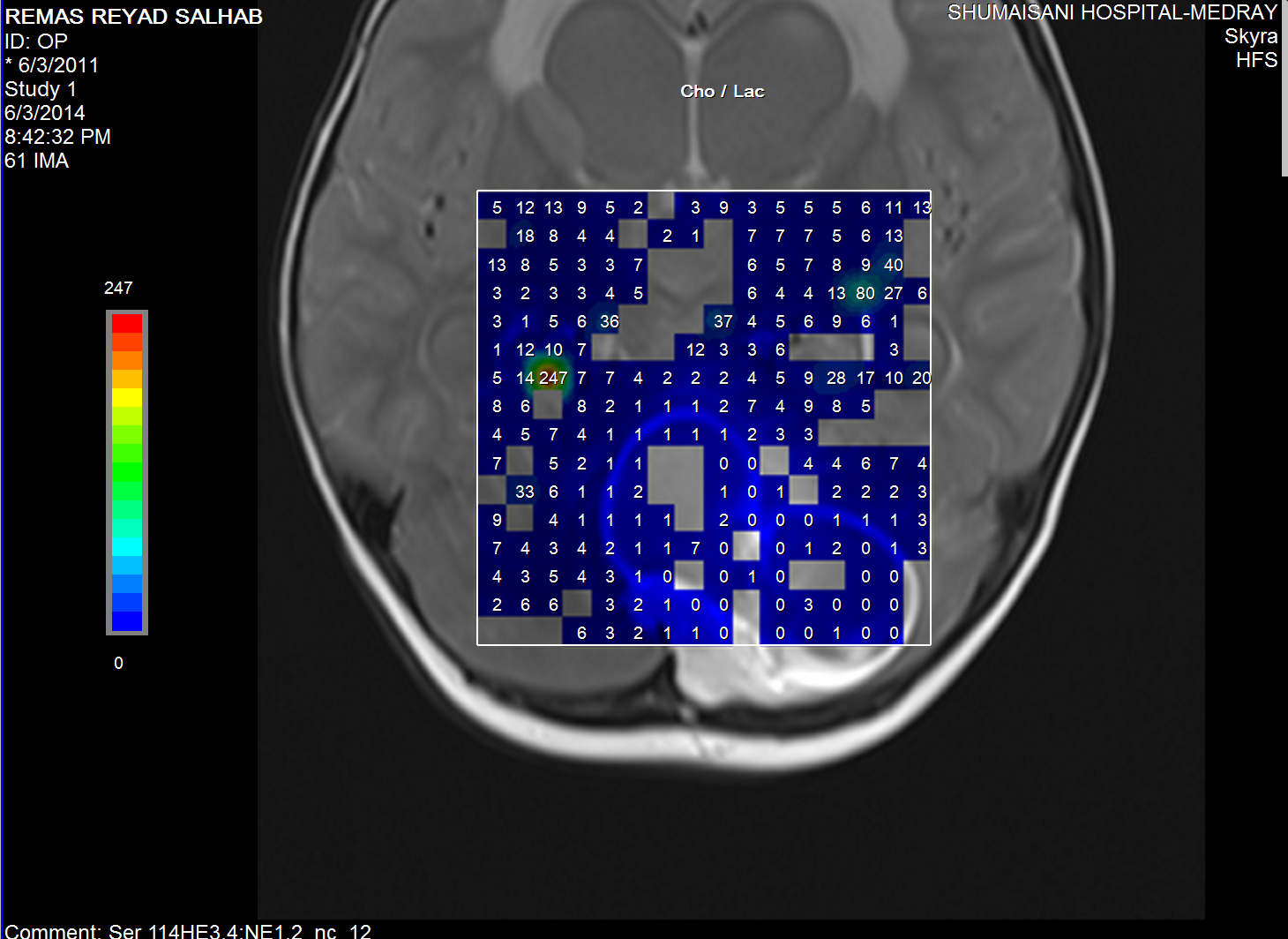
Choline/Lactate ratio.
The medulloblastoma shows low levels of NAA, as well as elevated
levels of Cho, lactate, and lipids, and peaks assigned to
taurine (Tau) and guanadinoacetate (Gua). Pilocytic astrocytomas
typically have low levels of Cr, as well as elevated lactate in
this example. As in adults, high-grade astrocytomas show
increased Cho compared to low grade, while NAA is absent in both
examples.
Comments
MRS is a helpful tool to diagnose the tumor before surgery.
Intraoperative MRI can confirm the radical resection before
closure and can show if there are any other events in the brain
nearby or remote from the site of surgery. It can predict the
postoperative care system of the patient.
|
 |
|